Introduction
At a biological level, there are two major ways light impacts plants:
- light is an energy source required to power any plant’s metabolism, and consequently any organisms that depend on plants as a food source (herbivores);
- light acts as a “maestro” to give plants cues about their surroundings and crucial information that helps them anticipate transitions between day and night, or transitions between seasons.
This translates into important changes at the level of plant size and shape (phenotypical), or physiological changes in terms of light-produced chemicals inside plants (phytocompounds) that are of interest for agricultural and agronomic purposes.
What is Photobiology?
Human beings have always been fascinated by sunlight.
Our days and clocks have been built around sunlight for centuries, and its pivotal influence has resulted in an ever-present yearning to study, understand and use its properties.
Egyptians used mirrors during the construction of pyramids and inadvertently discovered sunburning of the eye by ultraviolet exposure. Some of the oldest written records detail the dates of solar eclipses, such was the importance of sunlight from a very early stage in human history.
As a technology tool, light has had a significant impact in our society, far beyond even the societally-transformative invention of the light bulb.
For example, the discovery of luminescence (as in fluorescence and phosphorescence) caused a revolution in biotechnology by providing new, powerful tools for the visualization of molecular processes (as occurs in spectroscopy) and organisms as a whole (as in the bioimaging of, for example, luciferin, Aequorea victoria green-fluorescent protein, Förster resonance energy transfer (FRET) …).
Light has also played a fundamental role in environmental biology and ecology through the relationship with UV and ozone, as well as in medicine through observation of DNA damage by UV light. Of course, light is also fundamental to optics that are in everything from eyeglasses, grow lights and smartphones.
We’ve touched on the photo (light) aspect of photobiology, but what about biology? And where do the two intersect? After reading this article, you’ll have answers to these questions, and much more.
Photobiology is the study of interactions between light and living organisms.
Normally the light that is of specific interest for study is that of solar radiation reaching Earth in the ultraviolet, visible and infrared wavelengths. Photobiology is a large area of research that encompasses investigations into the nature of how organisms see (vision), how light can be harmful (as in plant phototoxicity), and how light produces energy (photosynthesis).
Photobiology has been pivotal in describing these vital biological processes, some of which we could not live without.
In plant biology, photobiology has explained how plants are able to discriminate between types of light as a function of spectrum, intensity or duration. The study of light interactions with molecules from living organisms (biomolecules) helps us understand how we can use light to improve plant cultivation.
At a nanoscopic level, molecules react with light when they absorb particles of light energy (known as photons) and go into an excited energy state. In order to regain energetic stability, molecules either react with particles around them, or they undergo conformational changes.
These two possible reactions lead us into the first and arguably most important application of photobiology, in the harvesting of energy by plants.
Photosynthesis
All living organisms need energy to keep themselves functioning. They can get energy from a wide variety of sources. Animals eat plants or other animals to absorb sugar, nutrients, protein, and fat. Fungi use decomposing matter to get their food.
Plants, on the other hand, get their energy from sunlight, CO2 absorbed in the atmosphere, and nutrients and water in the soil.
Light is the primary energy source for most plants and subsequently for most living organisms. It is essential to understand how the light-plant relationship is established and how plants convert light into usable/available and efficient energy. The light-plant relationship is crucial because the sustainability of the whole food chain depends on this initial energy production, the sequence of reactions called photosynthesis.
Photosynthesis is the process in which plants capture light and use this energy to produce sugar by consuming CO2 (carbon fixation) and releasing oxygen.
The light can come from the sun or artificial light, as long as it has the correct wavelength. The sugar produced is in the energy form most usable by a plant’s metabolism.
Plants capture most of the light in their leaves as well as in any chlorophyll-containing tissues. The light is received in the cells by a specialized cellular structure or organelle called a chloroplast. Chloroplasts are plant-specific structures and hold a high concentration of pigments, such as chlorophyll and carotenoid, that capture light. Once the incident light has been captured by the plant, a light-dependent reaction is carried out by a chain of complex enzymes embedded in membrane-bound compartments of the chloroplast called thylakoids.
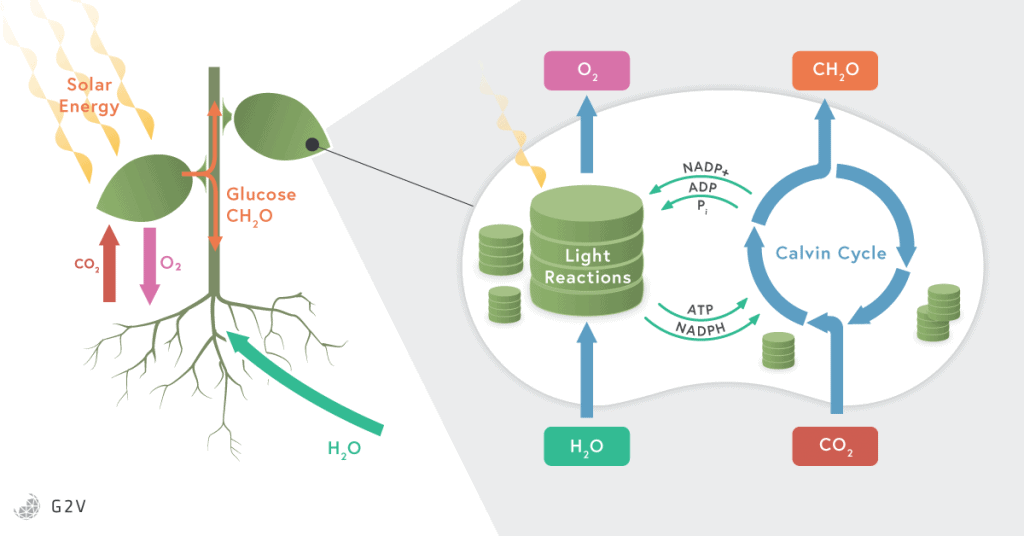
These light-dependent reactions are strongly influenced by large complexes of proteins and pigments, known as photosystems (PS) and light harvesting complexes (LHC). We’ve already mentioned chlorophyll and carotenoids, which are pigments inside the photosystems, and there are others (such as xanthophylls) that are also included in the photosystem complexes. To be more specific, the photosynthetic pigments are arranged in what’s called a light harvesting complexes surrounding photosystems I and II. These two photosystems are what allow electrons to be collected from the LHC, then passed through the different complexes to produce energy.
The pigments inside a chloroplast each have a different spectrum of light that they will absorb; in other words, each pigment has a different peak and range of wavelengths of light it will respond to. There are two types of chlorophyll, chlorophyll a and b, that have spectral absorptions shifted 20 nm from one another, which combine to allow a wider light absorption bandwidth by the plants. They complement each other.
Chlorophyll a, b and carotene are inserted in the thylakoid membrane. They have different absorption spectra in the red and blue and are directly responsible for the absorption of sunlight in plant leaves.
Looking at their chemical structure, these pigments have a hydrophobic (water-hating) phytol tail that allows them to be anchored in the membranes while the porphyrin ring (head) absorbs the light. Chlorophyll b has an aldehyde functional group, whereas chlorophyll has a methyl group. These key differences determine their different absorption spectra.
Because chlorophylls are abundant in plants and absorb mainly red and blue wavelengths, they reflect green light and make leaves appear green to human eyes.
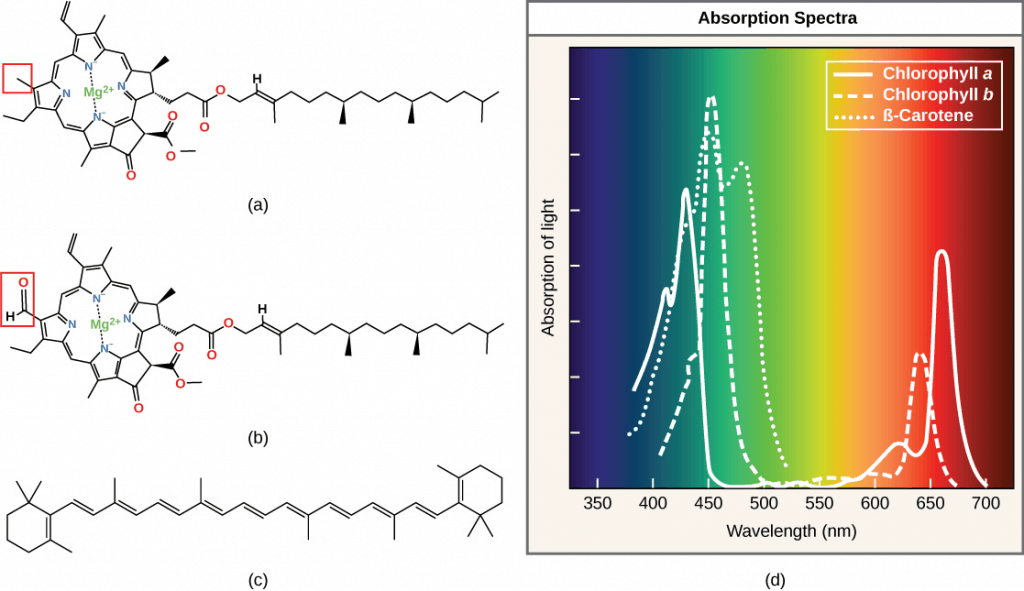
Image source: courses.lumenlearning.com/boundless-biology/chapter/the-light-dependent-reactions-of-photosynthesis
The process of photosynthesis happens in the following manner. The light incident on a plant excites electrons in chlorophyll. In order to regain energetic stability and replace its lost electrons, chlorophyll then pulls electrons from water in the reaction center. When water loses an electron, it splits apart into hydrogen and the oxygen that we breathe.
The electrons excited in chlorophyll by the absorption of light are passed along into different enzymatic complexes, also located in the chloroplast (in the thylakoid membrane) to produce NADPH and ATP (the primary energy “bricks”). NADPH and ATP are directly used in enzymatic reactions throughout all plant cells, but most importantly to perform the next phase of photosynthesis.
This phase involves CO2 assimilation into glucose (known as the Calvin Cycle) that is used directly to form cellulose, lipids, or proteins; or stored as the starch in leaves, in tubers such as potatoes, in roots such as carrots, or in the seeds. Glucose is a more stable form of energy than ATP and NADH and can be stored in long polymeric carbohydrate structures such as starch.
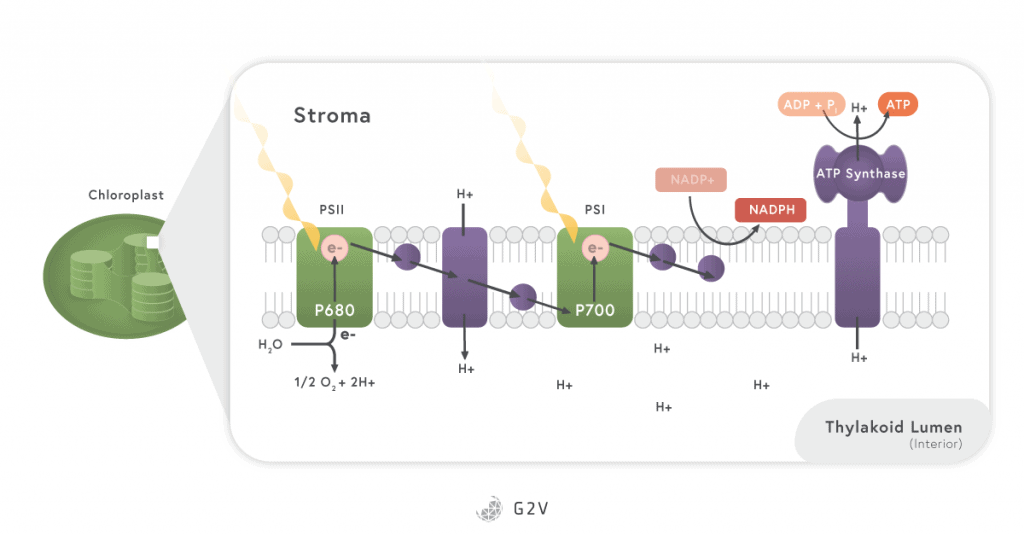
Image data source: khanacademy.org/science/biology/photosynthesis-in-plants/the-light-dependent-reactions-of-photosynthesis/a/light-dependent-reactions
It is well known that red light (625 nm – 675 nm) and blue light (450 nm – 485 nm) drive the photosynthesis process by causing excitation of chlorophyll within plant leaves.
Photosynthesis can be measured through different methods such as the net assimilation rate, which is the increase in dry weight of the leaf area.
In some species (i.e. radishes, cucumbers, peppers and lettuce), increasing certain flux levels of blue light can improve photosynthetic efficiency by 10 to 25%.
Light Stress in Plants
Different pigments are optimally arranged in light-harvesting complexes surrounding photosystems I and II (PSI and PSII). While chlorophyll is responsible for the primary absorption, the other pigments contribute in different ways. The carotenoids and xanthophylls support the chlorophyll by absorbing any excess light that could cause the system to be inhibited through a phenomenon called photoinhibition.
Photoinhibition is a broad term describing the decrease in the efficiency of photosynthesis when plants are exposed to an excess of light. It usually happens when PSII is saturated with photons. Carotenoids and xanthophylls help prevent this damage from occurring.
This protection system of carotenoid and xanthophylls mentioned above has limitations, however, so photoinhibition can and does still occur when the intensity of light is high enough.
When photoinhibition occurs, it can lead to a decrease in CO2 assimilation and plant growth. Any excess of light absorbed by the plant must be regulated by being re-emitted as fluorescence or heat (non-photochemical quenching). This regulation comes at a cost, specifically, an increase in water absorption by the plant. You can already see the different balancing needs of a plant that can change very rapidly.
Thus, to maximize plant growth and minimize plant stress, it is important to not only deeply understand a plant’s requirements, but be able to quickly make adjustments to the light applied.
The carotenoids and xanthophylls can only protect so far, and the plant grower needs to know when their light intensity is too high. When the light intensity is too high, the fluorescence or thermal dissipation of energy is insufficient, resulting in pigment degradation and accumulation of reactive oxygen species (ROS). ROS are molecules and free radicals that can degrade cell structures (for example mutations in a DNA sequence) when they are in abundance.
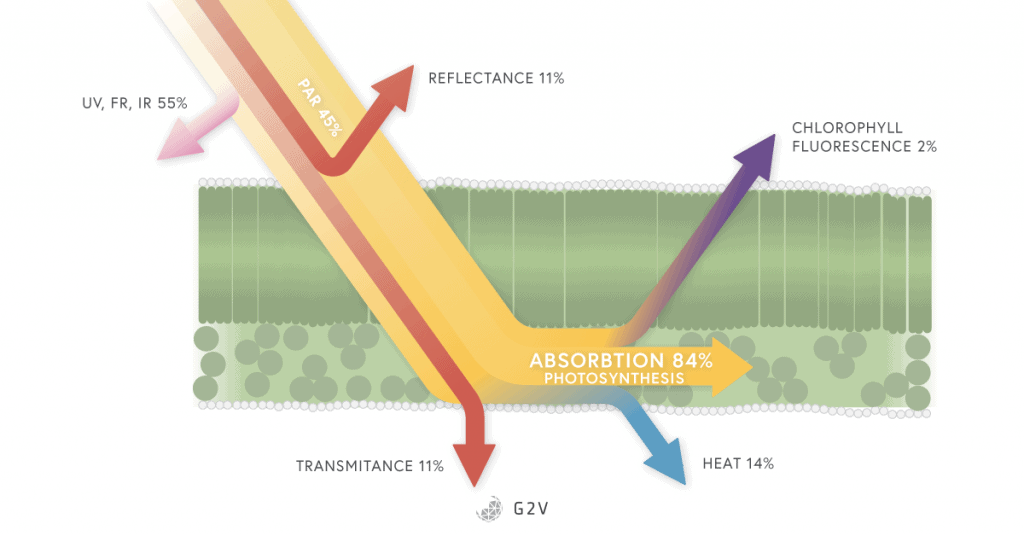
Image data source: currentscience.ac.in/Volumes/114/06/1333.pdf
Chlorophyll fluorescence can be used as an indicator of plant stress because environmental stresses (temperature or light intensity) can reduce the ability of a plant to metabolize normally.
This can mean an imbalance between the absorption of light energy by chlorophyll and the use of energy in photosynthesis (not all the light absorbed will be used in photosynthesis and so we are losing energy, which happens if we are not using the correct lighting system).
Energy absorbed by chlorophyll can be dissipated via photochemistry (photosynthesis), by heat or carotenoids activation (non-photochemical quenching), or as fluorescence. The competition between these processes allows us to determine the efficiency of PSII.
We can easily measure fluorescence with a chlorophyll fluorometer. We don’t need a laboratory or complex experiments. It can be done in the field with a portable fluorometer and it’s instantaneous. It’s a non-invasive measurement and we don’t need to sacrifice leaves or plants to get a measurement. That is why it is such a powerful variable.
To do so we can measure the Fv / Fm ratio (known as the quantum efficiency of PSII, or photochemistry efficiency). After dark adaptation (which takes anywhere from a few minutes to overnight), and under a very low light intensity (for example, the light at dawn), the minimum fluorescence is measured.
Fluorescence by chlorophyll happens when excited electrons regain stability. In other words, fluorescence happens when chlorophyll absorbs light and does not pass those excited electrons onto the light-harvesting complex. Following exposure to low light, the leaf is then submitted to intense light that will saturate and close the light harvesting complex (containing chlorophyll). Under these conditions, the maximum fluorescence (Fm) can be measured. The difference between maximum and minimum fluorescence is Fv, the variable fluorescence.
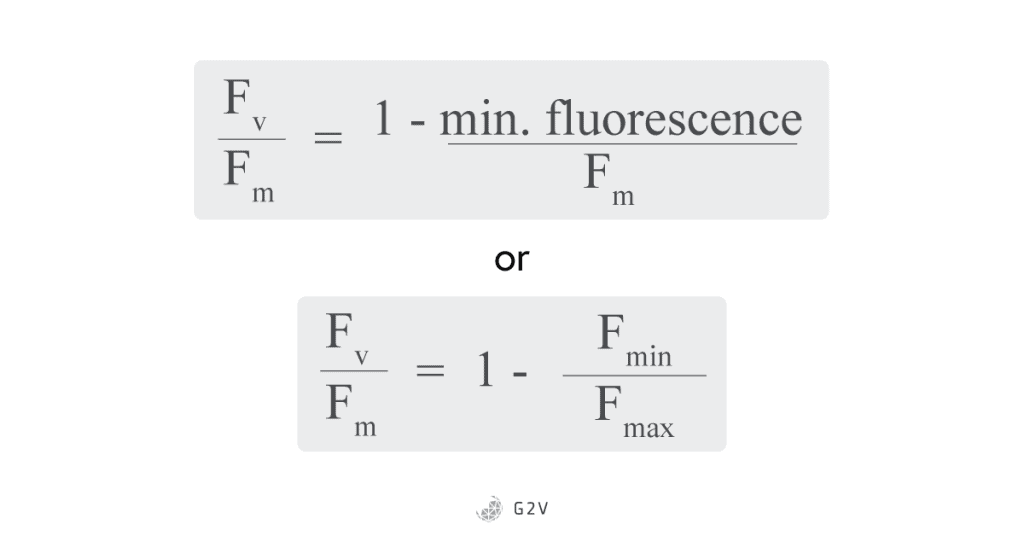
When the light harvesting centers are closed it means they are saturated and cannot pass any more electrons until they regain stability. This causes a decrease in quantum efficiency of PSII (Fv/Fm decrease).
The purpose of this behaviour (closing the light harvesting centers) is to avoid PSI photoinhibition (PSI lacks efficient repair mechanisms). It is easier for PSII to recover from photoinhibition so in order to prevent that, the flow of electrons to PSI is restricted by PSII.
The ratio of Fv/Fm represents the maximum conversion ratio (or maximum quantum efficiency) of light being usefully absorbed by the photosystems for photosynthesis. Another way to think of it is in this way: Fv indicates how much light can be absorbed by the photosystem before saturation. By dividing by Fm, we are normalizing the units to a fraction or percentage (i.e. quantum efficiency). The Fv/Fm ratio indicates how much light can be absorbed before it is lost through fluorescence (i.e. how much light is needed to saturate the light uptake of the photosystem).
The higher the Fv/Fm ratio, the greater the plant’s capacity for useful light absorption (plants are not easily saturated with light and fluorescing). When a plant is stressed, Fm decreases; that is, it can pass fewer electrons onto the light harvesting complex. A smaller Fm value results in a smaller Fv/Fm ratio (because the Fmin/Fmax value increases; see above equation).
A Fv/Fm ratio of 0.8 is considered an optimal value for most plants.
Chlorophyll molecules, when they have excited electrons that cannot be passed into the light-harvesting complex, re-emit light as fluorescence F or as heat Q.
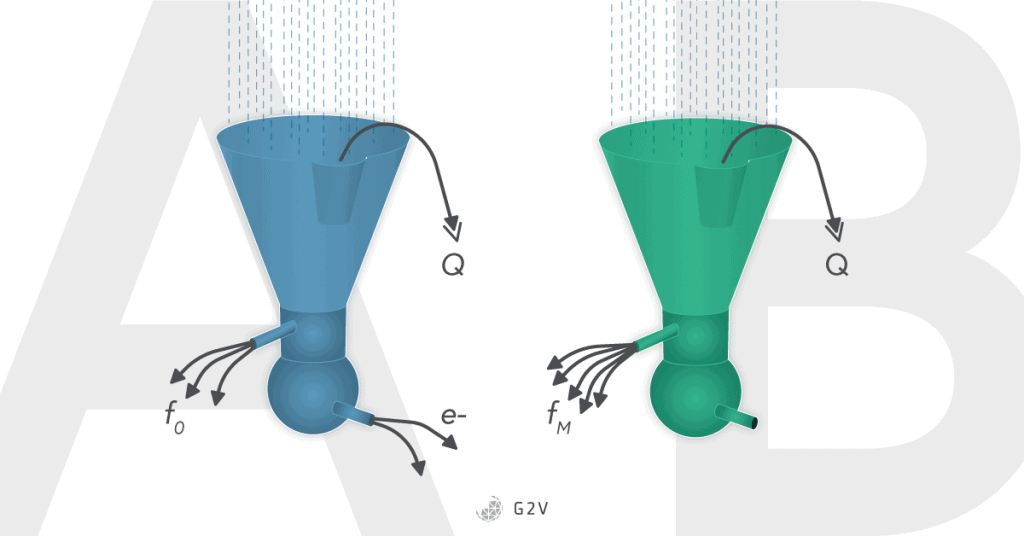
Light damage and light stress in plants are serious issues, but thankfully plants have a few mechanisms in place for defending themselves:
- The presence of carotenoids and xanthophylls that catch excess photons (mentioned above).
- The process of self-shading. For example, when chloroplasts move into a low absorbing position, or the leaf itself moves to decrease its light exposure.
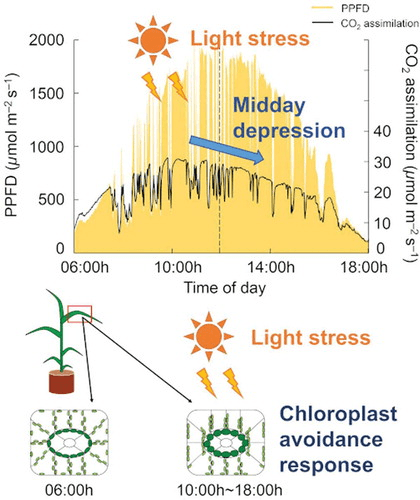
Image source: tandfonline.com/doi/full/10.1080/1343943X.2019.1673666
- The action of antioxidants (such as vitamin c and vitamin e) that capture free electrons and can thus mitigate the damage of ROS molecules and free radicals.
Whenever plants need to protect themselves from intense illumination, they are wasting energy that would otherwise go toward biomass and fruit production. Thus, light stress impacts the post‐harvest yields of crops.
Some producers will want to stress plants on purpose in order to have them produce compounds of interest that are generated under light stress conditions. For example, one might want to use UV-B to increase flavonoids quantities in fruit and berries, anthocyanin in apples and litchis or vitamin C in basil. These compounds, along with vitamin C, are major electrons scavengers and are produced by the plant to counteract the increased concentration of ROS under high light-intensity conditions.
We’ve talked about high light-intensities, but plants are stressed under low light conditions as well.
Low light intensity affects plant growth dramatically.
When photosynthesis is not fueled by an appropriate flux of photons, a plant’s ATP productivity (plant food production) is lowered. This lower energy production leads to shade avoidance symptoms (such as the elongation of stems and petioles, the stalks that attach stems to leaves), flower bud abortion, and inhibition of growth.
Finally, if low light conditions are prolonged enough, a program of leaf senescence is initiated, and the plant dies.
Photosynthetically Active Radiation (PAR)
As we discussed earlier, chlorophyll, carotenoids, and xanthophyll don’t absorb just any kind of light: they are specialized pigments with specific wavelength absorption bands. Photobiology researchers have come up with a broader term to refer to these specific wavelengths of light useful for photosynthesis: photosynthetically active radiation (PAR).
PAR is the part of the electromagnetic spectrum that is effective for photosynthesis, ranging from 400 nm to 700 nm.
It is a useful definition in photobiology because it refers specifically to the band of radiation crucial for energy production in plants. Measuring PAR will thus give a better indication of photosynthesis potential. The intensity/amount of PAR is measured by the Photosynthetic Photon Flux Density (PPFD) which is a quantification of the photons received by a surface for a given time (in units of µmol m-2 s-1). This quantity is important as photosynthesis is a quantum process where 8 to 12 photons are considered necessary for the incorporation of one CO2 molecule and the release of an O2 molecule.

Image data source: telec.co.za/forum/led-grow-lights-5/question/what-is-the-difference-between-ppf-and-ppfd-buyer-beware-10
PAR has a narrower range than the radiation of the sun that reaches the Earth’s ground and plants.
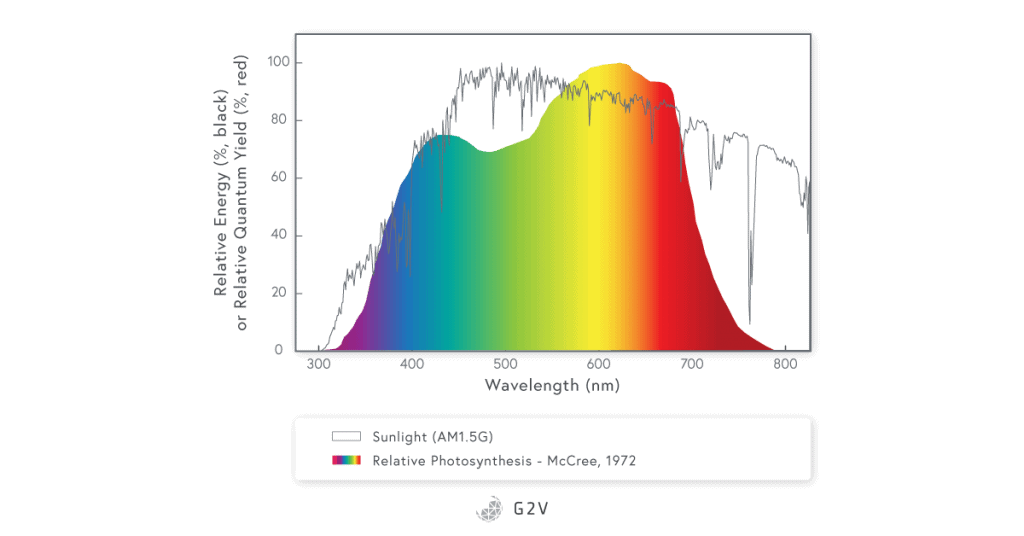
Image data source: sciencedirect.com/science/article/abs/pii/0002157171900227
The amount of PAR received by a plant could be higher than necessary or not optimum depending on the weather. There is therefore some benefit to using artificial light sources that are more stable and reliable. While there are a wide variety of light sources available for horticulture, fully-programmable LEDs make for one of the better options because of their efficiency, stability and tunability that is suitable for designing a plant-specific PAR spectrum.
It is very important to remember that the PAR spectrum as defined by McCree represents an average of 22 different plants and thus the PAR for a specific plant can be optimized by increasing or decreasing specific wavelengths as a function of the plant cultivated and the desired traits.
Indeed, the definition of PAR by McCree needs to be extended as we know now that radiation outside PAR wavelengths can improve photosynthesis. The range of photosynthetically active radiation should also be redefined for each crop.
The original McCree study reported the photosynthetically active radiation spectrum based on the quantum yield (the rate of photosynthesis per unit rate of absorption of light quanta) measured in 22 plant species using unique wavelengths (bandwidths of 10 nm to 40 nm at a time) in the range from 350 nm to 750 nm.
Wait, Aren’t Red and Blue the Best Colours for Plant Growth?
Yes, scientists agree that plants use red and blue radiation due to their high chlorophyll content, which absorbs blue and red light. It is also agreed upon that the use of radiation of both colours will enhance plant growth by making photosynthesis more efficient.
But, other wavelength regions are of major importance for plants. In fact, a lot of research has now shown that there is a synergy effect of light spectra on plant growth.
Green and yellow light are surprisingly also part of the PAR as they are also used during photosynthesis in interesting processes.
We tend to think that because the vast majority of plants on the planet look green, they reflect green radiation and thus have no use for it. Some studies, however, have revealed that green and yellow light also increase the net assimilation rate in cherry tomatoes, red leaf lettuce and cucumbers.
The influence of green and yellow light is not isolated to these specific species. Studies have shown that the plant canopy can absorb up to 80% of the green radiation received.
Other recent findings demonstrate that green radiation penetrates deeper than blue and red radiation into the leaf mesophyll (the inner tissue of a leaf) where the number of chloroplasts is higher than the leaf surface (a 10:1 ratio mesophyll chloroplasts to epidermis chloroplasts).
This higher concentration of chloroplasts results in a more efficient carbon fixation than that achieved using only red and blue radiation. Green light brings energy to deeper layers of cells in leaves or will be transmitted and distributed to other leaves deeper in the canopy. Green radiation also plays a role in providing a positional signal in addition to the quantity of blue, red and far red light that trigger the shade avoidance process.
Understanding the dynamics of light in the atmosphere, inside a group of plants and within a plant itself helps us to grow plants that are surrounded by others, and to apply light optimized for a specific plant population.
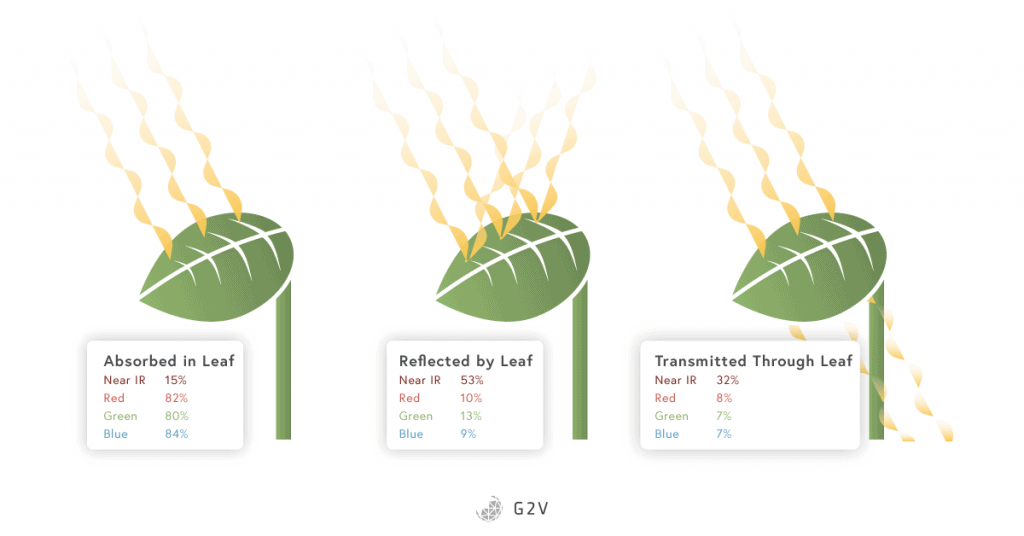
There is far more complexity to the optimal light for a plant’s food production than simply illuminating them in red and blue.
Choosing a grow light by taking into account how much of the PAR it can deliver, and how flexible it can be in adjusting the type of radiation delivered is crucial if you want to provide the best quality light to your plants.
Plant Light Perception
We’ve seen how plants absorb and use light for food production in photosynthesis. There are additional ways plants interact with light which are important for any master grower to consider, the first of which is plant perception.
Photomorphogenesis: Photoperiodism and Phototropism
Light is perceived by plants by a network of photoreceptors (also called pigments), which trigger developmental and environmental plant responses. This phenomenon is called photomorphogenesis. There are many different types of photomorphogenic responses. One example is a phototropic response, where a plant’s stem bends towards or away from a light source.
Another example is a photoperiodic response, which is a response to the length of the day and/or season(s) by modifying plant physiological processes like seed and bud dormancy, flowering and leaf maintenance.
Plants can track the time of the day and even changes between seasons. They have a memory of the day and night length like many species on earth – this is known as circadian rhythm.
A plant’s circadian rhythm is driven by the cellular expression of factors (transcription factors) that are expressed as a function of the day. There are day elements (or genes) that are repressing the night elements to allow day functions such as photosynthesis or starch production to be carried on during light exposure. When light intensity decreases and night sets in, evening elements are repressing the day elements to stop day-time activities and to carry on night activities such as starch conversion into glucose.
The two complexes (day elements and night elements) act in a feedback loop system to regulate a plant’s specific day and night activities. With this system, plants can anticipate events to come, like the transition between day and night. This is essential so plants can prepare for environmental changes like cold weather or periods without light.
On the other hand, some photoreceptors can track the origin of light and induce plant movement and growth in relation to the direction of the light in order to maximize light reception (positive phototropism) or to minimize it (negative phototropism). This is called phototropism and is regulated by photoreceptors called phototropins. These receptors absorb blue light which has the effect of changing their protein conformation. This conformational change in turn drives an accumulation of the hormone auxin at the opposite side of the light origin, or the shady side of the plant.
The accumulation of auxin leads to an elongation of the cells in the plant’s shady side, with the ultimate result of bending the stem toward light. Sunflowers are a beautiful example of plants following sunlight.
The phototropic response allows plants to optimize their exposure and carbon gain, to protect themselves from too much light and to find a light source when seedlings are germinating.
In plants, photoreceptors can be divided into 4 families each containing several members, each of which will be discussed in more detail below:
- Phytochromes (PhyA to PhyE)
- Cryptochromes (CRY1, CRY2 and CRY3)
- The Light-oxygen-voltage (LOV)-domain photoreceptor family
- Phototropins (PHOT1 and PHOT2)
- ZTL/FKF1/LKP2 group proteins
- UV-B resistance 8 (UVR8)
These photoreceptors sense all different kinds of light cues (intensity, spectra, photoperiod).
They all contain a chromophore, which is a part of a molecule sensitive to light (for example, the porphyrin ring in chlorophyll). The chromophore can be excited by light and allows a circulation of electrons which can lead to modification of a molecule’s conformation (as in dimerization, joining two into one, for example). The excitation of a chromophore can also allow electrons to simply jump to another molecule. This generally triggers an intracellular signaling cascade.
The intracellular signaling cascade is a series of reactions between molecules inside a cell that induces a response to a given stimulus. When the sun’s radiation reaches a plant cell, photoreceptors located on the leaf surface or inside the cells are activated by excitation of their chromophore part. This activates or represses different types of molecules that can regulate gene expression. This regulation of gene expression eventually leads to the degradation or production of new molecules that induce a response to this light radiation.
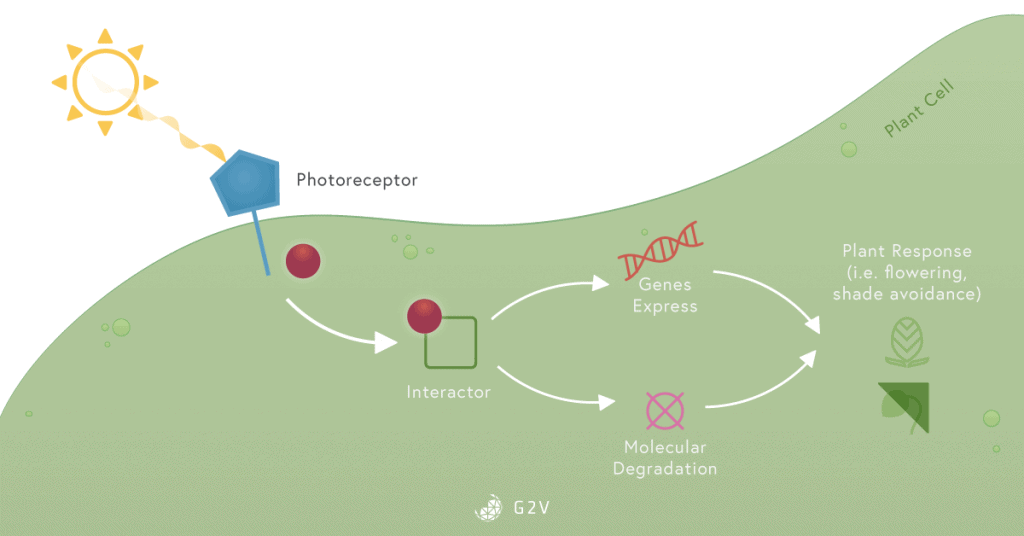
Phytochromes
Phytochromes are pigments that absorb light in the red and far-red region of the visible spectrum.
They regulate the synthesis of chlorophyll, the germination of seeds, the elongation of seedlings, the timing of flowering in adult plants, as well as the size, shape, number and movement of leaves.
Phytochromes are expressed across many tissues (flower, leaves, roots) and developmental stages (seed coat, cotyledons, inflorescence). Red light and far-red light can be applied to trigger phytochrome activity and regulate these physiological processes.
Phytochromes absorb red light at about 660 nm and far-red light at 730 nm, and they react to the ratio of red to far-red light intensity.
On a clear day around noon, the red to far-red ratio (R:FR) in natural daylight is close to 1. Essentially, this ratio signals if a plant is shaded by other plants in the vicinity. In this case where a plant is shaded by another, because far-red radiation is able to penetrate more deeply into the canopy (while red and blue radiation are absorbed), more far-red radiation will be detected by phytochromes (Pr to Pfr). This detection will trigger shade avoidance behavior such as stem elongation and the development of smaller leaves and branches. These responses are achieved through the redistribution of resources from the leaves to the stem.
Pfr conversion to Pr (R:FR low) increases apical dominance which decreases development of basal branching (apical dominance is when the terminal bud is inhibiting the growth of the secondary axillary bud by the controlled release of auxin hormone). Finally, the detection of far-red by the phytochrome affects leaf biomass and chlorophyll quantity. It also speeds up the transition to flowering which results in earlier seed production (this was originally observed in Arabidopsis thaliana). The red to far-red ratio (R:FR) could also play an important role in fruit quantity and morphology as studies have shown that in tomatoes FR increased (R:FR 0.88 to 0.7) can boost fruit biomass by up to 59%. However, there is still more work to be done as too few studies have investigated the dose effect of R:FR.
The red to far-red ratio is a good example of how plants glean detailed information from the spectrum with which they are illuminated.
The R:FR ratio is such an important signal that it can also affect a plant’s seed germination, where too much far-red radiation has been shown to inhibit germination in some species. Red and Far-Red radiation penetrate deeper into the soil than other radiation.
In an open space, red activates Phytochrome B (PhyB) and germination is initiated. If the far-red is high (under a dense canopy) some seeds can’t germinate. It’s therefore a very efficient way for plants to detect favorable conditions. However, in some species, Phytochrome A (PhyA) and PhyB are triggered under low fluence R light (PPFD < 10) to mediate germination. This is the case for Arabidopsis thaliana and tomatoes.
In addition to far-red radiation, the specific PhyA phytochrome is also activated by blue light in seedlings when uniteral blue light irradiation is low (observed in treatments as low as 0.5 PPFD).
It is important to note that phytochromes act as a dimer so we can find phytochromes in 3 different forms depending on which base molecules were joined into a pair. This gives plants several degrees of sensitivity to Red and Far-Red levels. This conformational change is a function of the light quantity (intensity), the ratio of R:FR as well as by temperature. For example, at low temperatures, Pfr reverses to Pr.
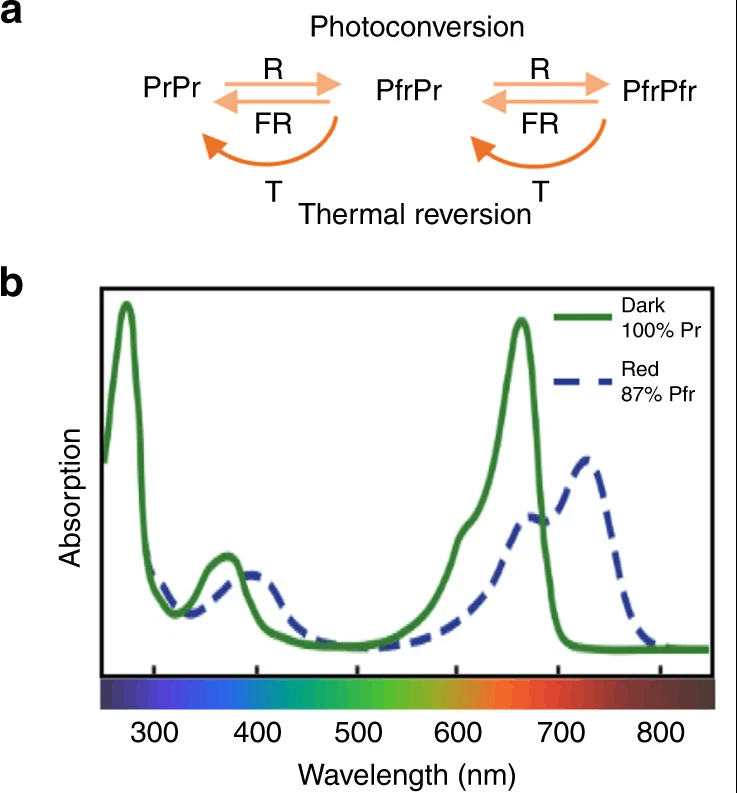
Image source: nature.com/articles/s41467-019-13045-0
Cryptochromes
Cryptochromes are pigments that are sensitive to blue light and UV-A.
They play a predominant function during de-etiolation (the transition to the greening stage after plant germination) (Cryptochrome-1 CRY1), in the photoperiodic control of flowering (Cryptochrome-2 CRY2), in the inhibition of the hypocotyl growth or in shade avoidance (CRY1 and CRY2).
Cryptochromes sense light with their chromophore which is a flavin adenine dinucleotide (FAD). Following light activation, they photodimerize (CRY1-CRY1) or oligomerize (CRY1-CRY2) and then bind to effectors (Cry-binding proteins) that will promote de-etiolation, transition to flowering (for CRY1) or senescence (for CRY2). It is important to note that CRY activation can lead to different plant responses depending on the plant species (CRY1 promotes flowering in soybean while CRY2 is responsible for flowering initiation in tomato and peas).
During the shade avoidance process in plants it has been established that under low blue light conditions, the interaction between CRY and PIFs is weakened, allowing PIFs (Phytochrome-Interacting Factors) to promote stem elongation.
Recently it has been demonstrated that cryptochromes are involved in different stress acclimation responses to events such as drought or hyperosmotic stress (where the plant’s surrounding liquid has a higher solute concentration) through modulation of CRY activity by a hormone-dependent signaling pathway. CRY activity could promote a protective effect during the above-mentioned abiotic stresses. Another protective effect CRY can promote is to induce hypocotyl growth through activation of the transcription factor PIF4 (PHYTOCHROME INTERACTOR FACTOR 4) under warm conditions, which would allow a better heat dissipation. Transcription factors are molecules which interact directly with genes to promote or inhibit their transcription. PIF4 here will directly interact with DNA coding for proteins involved in plant growth, and activate their production.
In other words, a plant’s light response is also modulated by its ‘’stress’’ state, emphasizing the importance of the environment on plant photobiology (see Phytochrome and temperature effects).
The LOV-domain photoreceptor family
The light, oxygen, or voltage (LOV) family of blue-light photoreceptors is a family of proteins present across all kingdoms of life (fungi, plants and bacteria) in which blue light photoexcitation of their LOV-domain lead to a biological signal (structural and dynamical changes and binding to other proteins).
Phototropin Photoreceptors
Phototropins are the first type of LOV-domain photoreceptors we’ll discuss. They are blue-light receptors controlling a range of responses to optimize the photosynthetic efficiency of plants. These responses include phototropism, light-induced stomatal opening, and chloroplast movement in response to changes in light intensity. Stomatal opening is when stomata (pores located in the plant epidermis), open to allow gas exchange: the absorption of CO2 and the release of O2.
This gas exchange is extremely important for energy production, as it allows CO2 uptake and its transformation into glucose following photosynthesis.
One of the most impressive phenomena associated with phototropin photoreceptors is their role in phototropism. Phototropism occurs when plants bend toward or away from light. This response is possible because part of the plant stem in the shaded side has increased growth.
This growth is due to a gradient of activation of a specific phototropin, PHOT1. The blue light crossing the stem section is refracted and the shaded side receives less light. The PHOT1 phototropin is not active in the dark, and since it plays a role in the degradation of the auxin hormone, there is a corresponding gradient in the auxin hormone. The accumulation of auxin in the shaded parts of the stem directs growth that bends a plant toward light.
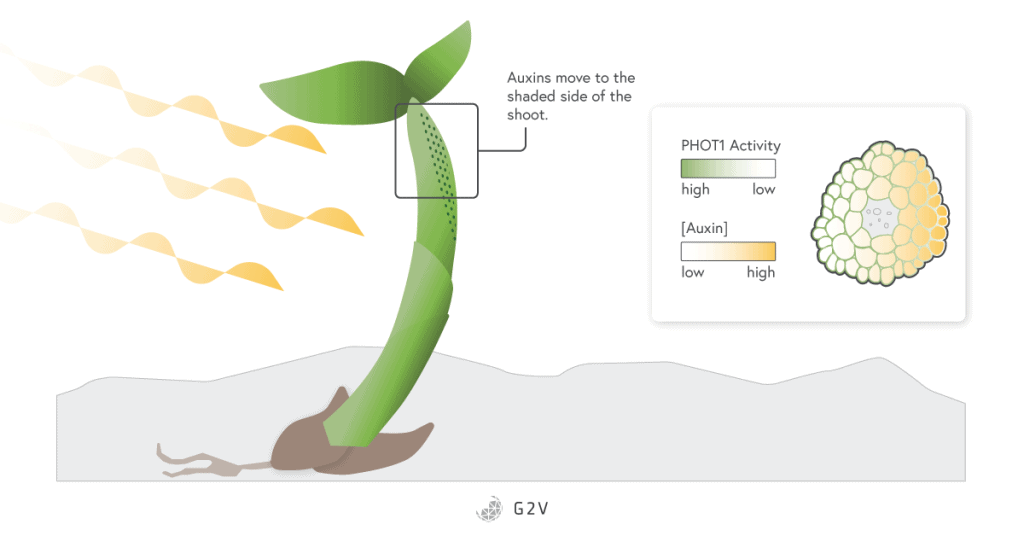
Zeitlupe (ZTL) Photoreceptors
The second type of LOV-domain photoreceptors are the Zeitlupe photoreceptors. ZEITLUPE, FLAVIN-BINDING KELCH REPEAT F-BOX 1, and LOV KELCH PROTEIN 2 (ZTL/FKF1/LKP2) group proteins are blue receptors involved in light-mediated protein degradation by ubiquitination (the attachment of a ubiquitin protein) during the circadian rhythm.
They are often described in terms of their domains, where domains are specific sequences of amino acids or DNA bases that have functional roles. All the Zeitlupe photoreceptors contain a light, oxygen, or voltage domain (LOV-domain) along with domains involved in protein stability (F-box and Kelch repeat domains). As a function of the time of the day, the Zeitlupe photoreceptors promote degradation or maintenance of circadian transcription factors and induce transitions in the day to dark cycle.
These photoreceptors act directly in response to light activation to regulate gene expression. Under blue light the Zeitlupe photoreceptors bind to transcription factors to stabilize them. In the dark this interaction is weakened, so the transcription factors are not protected and are therefore sent for degradation by the cell.
For example, ZTL late at night triggers the degradation of major components of the circadian rhythm (TOC1 and PRR5 proteins) that normally maintain the plant in an optimum physiological state in which to pass the night. With the late-night decrease of the concentration of these factors as a result of ZTL, the plant can then shift to day functions.
During each transition from day to night or night to day, the detection of light triggers or inhibits genes that control day (phototropism) or night events (cell wall biosynthesis, for example). All three LOV-containing F-box proteins (ZTL, FKF1, and LKP2) are involved in circadian clock events such as day/night transitions or day-length-dependent flowering.
There is still a huge gap in understanding how these proteins act at the level of the whole plant, and further research is needed to determine whether variations in blue light or other wavelengths influence the expression of these proteins.
UVR8 Photoreceptors
Recently, more attention has been given to a receptor in the ultraviolet (UV) wavelength region, UV RESISTANCE LOCUS8 (UVR8). UVR8 is a photoreceptor sensing UV-B radiation (with an absorption peak at 285 nm).
It is the most recent photoreceptor characterized. This is an interesting discovery because for a long-time plants were thought to only have receptors in the visible spectrum.
Around 7% of all solar radiation reaching earth is UV, and UV-B (280 nm to 315 nm) is the most harmful to plants, being capable of breaking a molecule’s chemical bonds resulting in the production of highly reactive molecules or ROS.
When UV-B reaches plant cells, UVR8 is activated and is involved in photomorphogenic response and defense mechanisms.
UVR8 is responsible for mitigating the effect of UV-B on plants. Upon activation, UVR8 triggers the production of compounds involved in oxidative stress protection such as phenols, terpenes or anthocyanins and can even enhance a plant’s defense against herbivores.
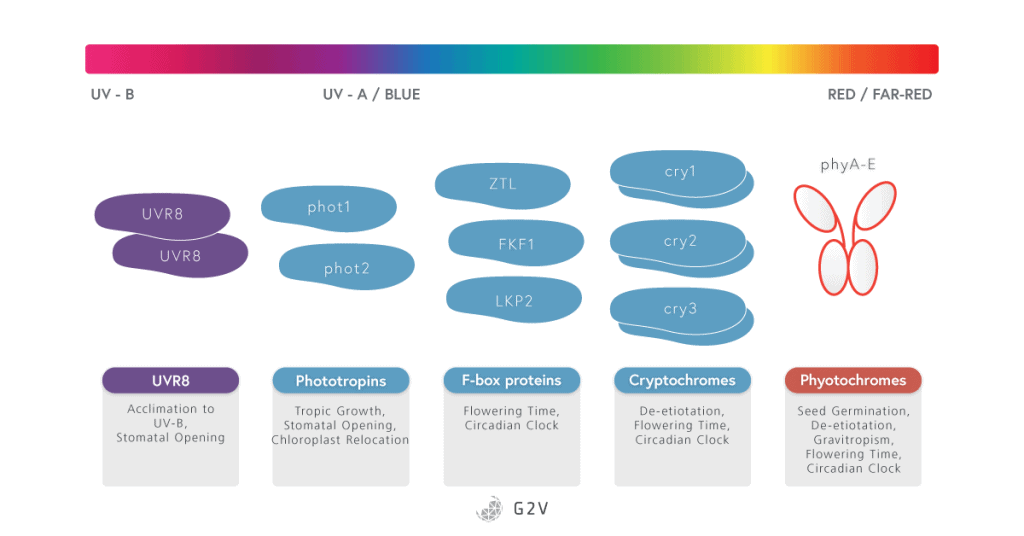
Image Data Source: doi.org/10.1016/j.semcdb.2019.03.007
Light Effect of Phytochemical Production
So far we’ve given an overview of the many different responses and pathways triggered and managed by plants interacting with light. While there is still much research that remains to be done, there are very practical outcomes that have already been gleaned through these insights into what’s happening inside each individual plant cell under illumination.
Researchers are now using these discoveries to better control and regulate the spectra under which they grow their plants, and looking at the specific effect it has on chemicals related to metabolic pathways (metabolites).
Studies have reported many interesting results regarding the increased production of secondary metabolites in response to the regulation of the light spectrum.
In Cannabis, for example, it was found that LED use could increase THC up to 38% compared to cannabis plants grown under high pressure sodium lamps (HPS). CBD was also increased by up to 35% under LED compared to HPS.
THC and CBD are phyto-compounds that have therapeutic values including anti-inflammatory and analgesic properties and can also be used to suppress vomiting, nausea and appetite. An increase from 15 to 32% in the THC content of Cannabis leaf and flowers was recorded after their exposure to UV-B radiation.
In basil, a properly matched light spectrum increased antioxidant capacity, phenolics and flavonoids by up to 16.3%, 28.8% and 41%, respectively, while reducing concentration of harmful compounds like nitrate by up to 41.6% showing that volatile compounds can also be manipulated by light.
Plant Disease Prevention
One of the interesting findings from testing specific light recipes is the effect of light on protection against disease.
Promising results have been shown on strawberries, tomatoes, cucumbers and peppers, all of which are especially affected by fungi in greenhouses.
Pathogens such as fungus (grey mold, powdery mildew) can cause great losses in crop production (10-15% yield loss in North America, according to the British Columbia Ministry of Agriculture).
It has been reported that UV-B light suppresses powdery mildew infection in strawberries by stimulating genes associated with disease resistance. Grey mold development on tomato leaves was suppressed by 63% with violet light application.
Light application could become a regular practice in the future, allowing the control of pathogen infections without using chemical methods.
It is extremely important to remember that all of the photoreceptors discussed in this article act in synergy to transmit information to the plant about the ambient/environment light conditions.
Because of this array of photoreceptors, plants can detect light from UV to IR, not only determining their intensities, but also sending signals about the time of exposure. Some photoreceptors are also sensitive to temperature. Plants can then sense and adjust to light changes such as the day/night transition. These light-sensitive adaptations can range from physiological to morphological adjustments.
Plants can elongate when they need to compete with other plants to reach sunlight on top of the canopy, or they can move their chloroplasts and leaves to avoid light damage at the cost of biomass production. Ultimately, plants thrive under optimum conditions of spectra, intensity and photoperiod exposure.
Ideally, you will want to adjust the amount, spectrum and time exposure of light according to the cultivar used (depending specifically upon general plant morphology as well as the quantity and type of photoreceptors), the stage of development (seedling to flowering), and the environment of cultivation (CO2 content, temperature, humidity). All of these parameters are essential for light use optimization.
For example:
- An increase in red light for certain plants (tomatoes, lettuce) can boost germination (helping seeds with lower storage reserves to germinate faster or, if used in reverse, to reduce the germination rate).
- A change in the photoperiod to induce flowering (for example, a transition from long 16-hour light days to shorter 12-hour light days to induce flowering in cannabis or chrysanthemum) or encourage leaf growth (cannabis).
There is some level of redundancy and cross talk in the light sensing and signaling pathways that make LED fine tune programming necessary to maintain desired crop qualities.
Frequently Asked Questions
Q: What is the value of variable spectra – what does it do for me?
Answer: Variable spectrum lighting can improve your plant growth considerably.
When you control the spectra of your light you can apply light best suited for your plant and save energy by not generating any light your plant doesn’t need.
By controlling the amount of each wavelength, an increase in yield up to 34% was reported in lettuce cultivation. In strawberries, maximum fruit production increased by 66% when the optimum level of red, blue and white light was attained. There is clearly a value in being able to adjust the light as a function of your crop species and also as a function of their growth stage.
As we explain in the article, seedlings have different needs (low intensity, more red) compared to mature or flowering plants.
Q: Can artificial lighting be better than the sunlight?
Answer: Since the start of agriculture, people have relied upon sunlight to grow their crops, and it still does a good job. The challenge is that sunlight is just not always available. Crops depend on seasons and weather, and during winter it’s simply not possible to grow outside.
Horticultural lighting is stable and reliable in intensity. With advanced lighting technology and research into photobiology, we can now design them with the perfect spectra and at the same time remove harmful radiation such as UV.
Q: What is the perfect spectrum for plant cultivation?
Answer: It is well known now that plants perform at their best under some ratio of blue and red.
Blue will encourage chlorophyll light absorption, photosynthesis, and growth while red radiation will also promote photosynthesis, growth and elongation.
However, UV-B, Green, yellow and IR can have various positive effects that can’t be neglected. Green penetrates deeper into the canopy while UV can trigger the production of specific metabolites that can have agronomic interest. Keep in mind that each plant has its preferred spectrums and that a full programmable lighting system will be the best at providing the best spectra throughout the life of your plants.
Q: How much light do plants need?
Answer: Usually, the light intensity required by a plant depends on the stage of growth and the plant species.
Sunlight intensity is between 900 and 1500 PPFD (micromoles per square meter per second).
Seedlings and small plants require between 100 and 200 PPFD. During vegetative growth, 400 and 600 PPFD is good for the development of the canopy. For flower and fruit development you can increase light intensity to 900 PPFD.
After 900 PPFD most plants will be limited by the amount of CO2 in the atmosphere and they won’t be able to use all the light. Every light intensity change should be made gradually so you don’t stress your plants and you can see what is the maximum limit specific to your plants.
Q: Can we grow plants under continuous light?
Answer: It is possible to grow certain plants under 24-hour light. But most plants will require a dark period. In the dark, they can recover, allocate resources for tissue reparation and prepare for the following day. Also, most importantly, some plants require periods of darkness to initiate flowering.
Some growers will grow their plants under a 24-hour light regime to a certain stage of growth and then add a darkness hour to trigger flowering. Most of the plants are grown under 12 to 18-hour light which leaves between 12 and 6-hours of dark.
Summary Points
- Light is the energy source for photosynthetic organisms such as plants or algae.
- Photons from light drive the photosynthesis reaction that allows the storage and the conversion of light energy into the form of glucose made from CO2 assimilation
- Plants can detect several light parameters through their very sophisticated photoreceptors such as wavelength, exposure time or light intensity, which is why quality lighting is essential to grow plants
- Inappropriate amounts or types of radiation (UV) can stress plants and even kill them
- For every plant, every species, and at each stage of development there is an optimal light condition
- Plants have evolved to recognize, anticipate and adapt to light changes.
- Light variations are translated into morphological and physiological outcomes in plants that are essential for their survival:
- Biomass
- Metabolites (vitamins, terpenes, anthocyanin…)
- Morphology
- Flowering
- Fruit production